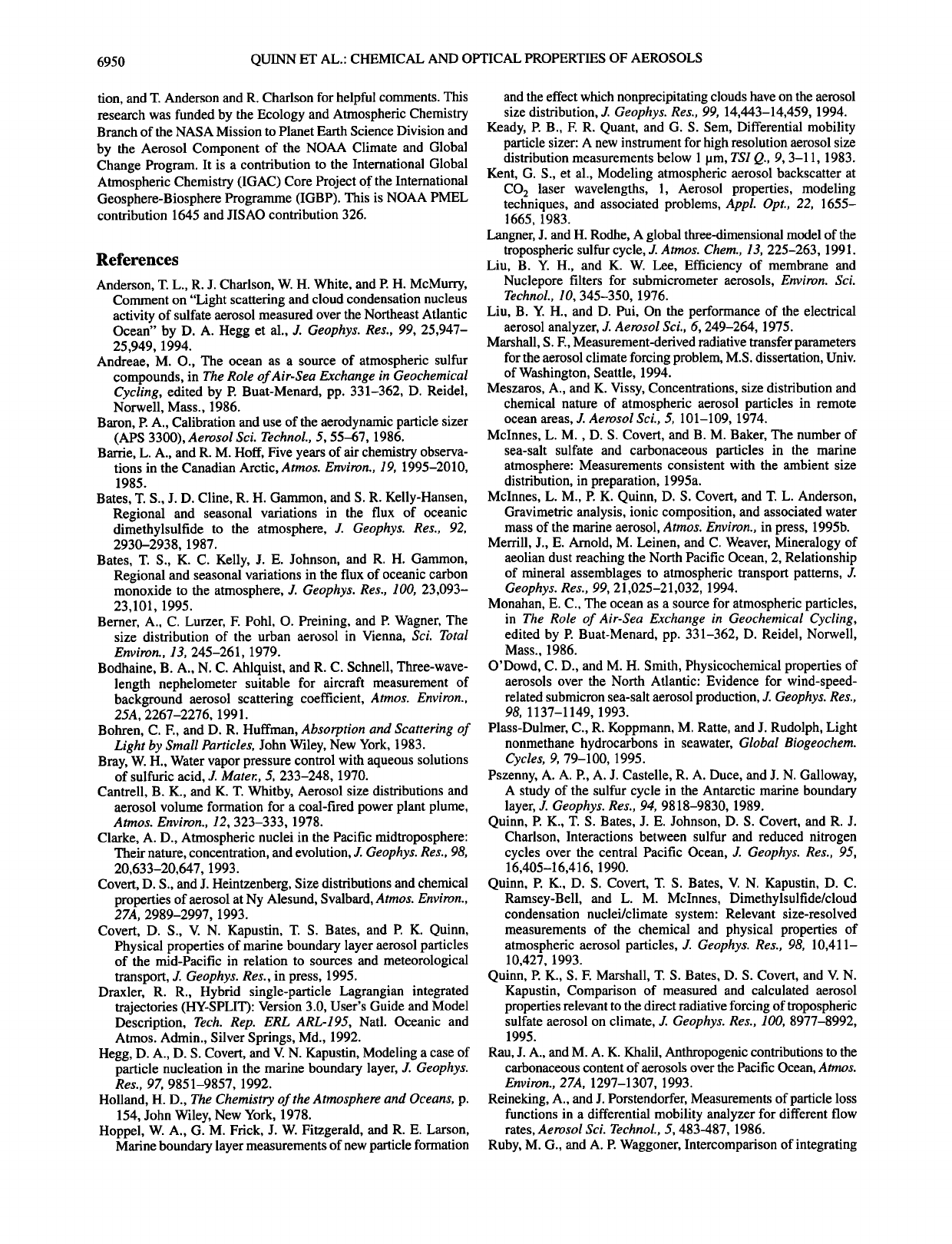
6950 QUINN ET AL.: CHEMICAL AND OPTICAL PROPERTIES OF AEROSOLS
tion, and T. Anderson and R. Charlson for helpful comments. This
research was funded by the Ecology and Atmospheric Chemistry
Branch of the NASA Mission to Planet Earth Science Division and
by the Aerosol Component of the NOAA Climate and Global
Change Program. It is a contribution to the International Global
Atmospheric Chemistry (IGAC) Core Project of the International
Geosphere-Biosphere Programme (IGBP). This is NOAA PMEL
contribution 1645 and JISAO contribution 326.
References
Anderson, T. L., R. J. Charlson, W. H. White, and P. H. McMurry,
Comment on "Light scattering and cloud condensation nucleus
activity of sulfate aerosol measured over the Northeast Atlantic
Ocean" by D. A. Hegg et al., J. Geophys. Res., 99, 25,947-
25,949, 1994.
Andreae, M. O., The ocean as a source of atmospheric sulfur
compounds, in The Role of Air-Sea Exchange in Geochemical
Cycling, edited by P. Buat-Menard, pp. 331-362, D. Reidel,
Norwell, Mass., 1986.
Baron, P. A., Calibration and use of the aerodynamic particle sizer
(APS 3300), Aerosol Sci. Technol., 5, 55-67, 1986.
Barfie, L. A., and R. M. Hoff, Five years of air chemistry observa-
tions in the Canadian Arctic, Atmos. Environ., 19, 1995-2010,
1985.
Bates, T. S., J. D. Cline, R. H. Gammon, and S. R. Kelly-Hansen,
Regional and seasonal variations in the flux of oceanic
dimethylsulfide to the atmosphere, J. Geophys. Res., 92,
2930-2938, 1987.
Bates, T. S., K. C. Kelly, J. E. Johnson, and R. H. Gammon,
Regional and seasonal variations in the flux of oceanic carbon
monoxide to the atmosphere, J. Geophys. Res., 100, 23,093-
23,101, 1995.
Bemer, A., C. Lurzer, F. Pohl, O. Preining, and P. Wagner, The
size distribution of the urban aerosol in Vienna, Sci. Total
Environ., 13, 245-261, 1979.
Bodhaine, B. A., N. C. Ahlquist, and R. C. Schnell, Three-wave-
length nephelometer suitable for aircraft measurement of
background aerosol scattering coefficient, Atmos. Environ.,
25A, 2267-2276, 1991.
Bohren, C. F., and D. R. Huffman, Absorption and Scattering of
Light by Small Particles, John Wiley, New York, 1983.
Bray, W. H., Water vapor pressure control with aqueous solutions
of sulfuric acid, J. Mater., 5, 233-248, 1970.
Cantrell, B. K., and K. T. Whitby, Aerosol size distributions and
aerosol volume formation for a coal-fired power plant plume,
Atmos. Environ., 12, 323-333, 1978.
Clarke, A.D., Atmospheric nuclei in the Pacific midtroposphere:
Their nature, concentration, and evolution, J. Geophys. Res., 98,
20,633-20,647, 1993.
Covert, D. S., and J. Heintzenberg, Size distributions and chemical
properties of aerosol at Ny Alesund, Svalbard, Atmos. Environ.,
27A, 2989-2997, 1993.
Covert, D. S., V. N. Kapustin, T. S. Bates, and P. K. Quinn,
Physical properties of marine boundary layer aerosol particles
of the mid-Pacific in relation to sources and meteorological
transport, J. Geophys. Res., in press, 1995.
Draxler, R. R., Hybrid single-particle Lagrangian integrated
trajectories (HY-SPLIT): Version 3.0, User's Guide and Model
Description, Tech. Rep. ERL ARL-195, Natl. Oceanic and
Atmos. Admin., Silver Springs, Md., 1992.
Hegg, D. A., D. S. Covert, and V. N. Kapustin, Modeling a case of
particle nucleation in the marine boundary layer, J. Geophys.
Res., 97, 9851-9857, 1992.
Holland, H. D., The Chemistry of the Atmosphere and Oceans, p.
154, John Wiley, New York, 1978.
Hoppel, W. A., G. M. Frick, J. W. Fitzgerald, and R. E. Larson,
Marine boundary layer measurements of new particle formation
and the effect which nonprecipitating clouds have on the aerosol
size distribution, J. Geophys. Res., 99, 14,443-14,459, 1994.
Keady, P. B., F. R. Quant, and G. S. Sem, Differential mobility
particle sizer: A new instrument for high resolution aerosol size
distribution measurements below 1 pm, TSI Q., 9, 3-11, 1983.
Kent, G. S., et al., Modeling atmospheric aerosol backscatter at
CO 2 laser wavelengths, 1, Aerosol properties, modeling
techniques, and associated problems, Appl. Opt., 22, 1655-
1665, 1983.
Langner, J. and H. Rodhe, A global three-dimensional model of the
tropospheric sulfur cycle, J. Atmos. Chem., 13, 225-263, 1991.
Liu, B. Y. H., and K. W. Lee, Efficiency of membrane and
Nuclepore filters for submicrometer aerosols, Environ. Sci.
Technol., 10, 345-350, 1976.
Liu, B. Y. H., and D. Pui, On the performance of the electrical
aerosol analyzer, J. Aerosol Sci., 6, 249-264, 1975.
Marshall, S. F., Measurement-derived radiative transfer parameters
for the aerosol climate forcing problem, M.S. dissertation, Univ.
of Washington, Seattle, 1994.
Meszaros, A., and K. Vissy, Concentrations, size distribution and
chemical nature of atmospheric aerosol particles in remote
ocean areas, J. Aerosol Sci., 5, 101-109, 1974.
Mclnnes, L. M., D. S. Covert, and B. M. Baker, The number of
sea-salt sulfate and carbonaceous particles in the marine
atmosphere: Measurements consistent with the ambient size
distribution, in preparation, 1995a.
McInnes, L. M., P. K. Quinn, D. S. Covert, and T. L. Anderson,
Gravimetric analysis, ionic composition, and associated water
mass of the marine aerosol, Atmos. Environ., in press, 1995b.
Merrill, J., E. Arnold, M. Leinen, and C. Weaver, Mineralogy of
aeolian dust reaching the North Pacific Ocean, 2, Relationship
of mineral assemblages to atmospheric transport patterns, J.
Geophys. Res., 99, 21,025-21,032, 1994.
Monahan, E. C., The ocean as a source for atmospheric particles,
in The Role of Air-Sea Exchange in Geochemical Cycling,
edited by P. Buat-Menard, pp. 331-362, D. Reidel, Norwell,
Mass., 1986.
O'Dowd, C. D., and M. H. Smith, Physicochemical properties of
aerosols over the North Atlantic: Evidence for wind-speed-
related submicron sea-salt aerosol production, J. Geophys. Res.,
98, 1137-1149, 1993.
Plass-Dulmer, C., R. Koppmann, M. Ratte, and J. Rudolph, Light
nonmethane hydrocarbons in seawater, Global Biogeochem.
Cycles, 9, 79-100, 1995.
Pszenny, A. A. P., A. J. Castelie, R. A. Duce, and J. N. Galloway,
A study of the sulfur cycle in the Antarctic marine boundary
layer, J. Geophys. Res., 94, 9818-9830, 1989.
Quinn, P. K., T. S. Bates, J. E. Johnson, D. S. Covert, and R. J.
Charlson, Interactions between sulfur and reduced nitrogen
cycles over the central Pacific Ocean, J. Geophys. Res., 95,
16,405-16,416, 1990.
Quinn, P. K., D. S. Covert, T S. Bates, V. N. Kapustin, D.C.
Ramsey-Bell, and L. M. Mclnnes, Dimethylsulfide/cloud
condensation nuclei/climate system: Relevant size-resolved
measurements of the chemical and physical properties of
atmospheric aerosol particles, J. Geophys. Res., 98, 10,411-
10,427, 1993.
Quinn, P. K., S. F. Marshall, T S. Bates, D. S. Covert, and V. N.
Kapustin, Comparison of measured and calculated aerosol
properties relevant to the direct radiative forcing of tropospheric
sulfate aerosol on climate, J. Geophys. Res., 100, 8977-8992,
1995.
Rau, J. A., and M. A. K. Khalil, Anthropogenic contributions to the
carbonaceous content of aerosols over the Pacific Ocean, Atmos.
Environ., 27A, 1297-1307, 1993.
Reineking, A., and J. Porstendorfer, Measurements of particle loss
functions in a differential mobility analyzer for different flow
rates, Aerosol Sci. Technol., 5, 483-487, 1986.
Ruby, M. G., and A. P. Waggoner, Intercomparison of integrating